ERL X-ray Science Workshop 1 Abstracts
ERL Project Summary and Characteristics
Sol M. Gruner Cornell High Energy Synchrotron Source, Cornell University Physics Department, Cornell University The principle of operation of an Energy Recover Linac (ERL) is explained. The status and goals of the Cornell ERL project are summarized. Relevant characteristics of a 5 GeV ERL of the type we hope to build are described and some example experiments are given.Characteristics and Properties of the Jefferson Lab ERL and Applications of the High Brightness 4th Generation Light Source for High Pressure Research
Gwyn P. Williams & the JLab Team Jefferson Laboratory Jefferson Lab operates a fourth generation accelerator-based light source. The facility is based on an Energy Recovered Linac (ERL)1, which has a significant advantage in brightness over a conventional electron storage ring (synchrotron) source. Both terms contributing to the brightness are enhanced. The power is enhanced by multiparticle coherent effects2, while the source size is smaller because the horizontal emittance is approximately equal to the vertical emittance (round beams). This type of source has additional advantages in that the bunch lengths are in the 100's of femtosecond range, allowing ultrafast phenomena to be studied in the time domain. The JLab facility incorporates 3 sources: (1). A 10 kW av. power 1-14 micron tunable Infrared Free Electron Laser (IRFEL) with energies of 120 microJoules per pulse (close to 1030 photons/sec): (2). A 1 kW av. power 250 nm to 1 micron UVFEL with up to 25 microJoules per pulse: and (3). A 0.1 to 5 THz source3 with 1 microJoule per pulse. All 3 sources can operate at pulse repetition frequencies up to 75 MHz and pulse lengths in the range 250 - 2000 femtoseconds. We will present details of the source, and will make comparisons with storage ring sources and x-ray FELs. We will then discuss applications of this type of source for high pressure research in the far-IR, one example being the opportunity to measure the Drude absorption at long wavelengths, which signals the onset of conductivity. Here, some of the key issues have to do not just with brightness, but with noise. There is also the possibility to drive out-of-equilibrium phenomena with multiple photon beams. Finally we will briefly mention the characteristics of Jefferson Lab's a 6GeV accelerator, which is capable of producing sub-picosecond x-rays with a brightness comparable to dipole radiation at a storage ring. References: 1. G.R. Neil et al, Phys. Rev. Let. 84, 662 (2000) 2. C. J. Hirschmugl, M. Sagurton and G. P. Williams, Physical Review A44, 1316, (1991) 3. G.L. Carr, et al, Nature 420 153 (2002) This work supported by the Office of Naval Research, the Joint Technology Office, the Commonwealth of Virginia, the Air Force Research Laboratory, The US Army Night Vision Lab, and by DOE under contract DE-AC05-84ER40150.High-Pressure Synergetic Consortium -- A New Approach to High-Pressure Research at Synchrotron Facilities
Ho-kwang Mao Geophysical Laboratory, Carnegie Institution of Washington We have witnessed an unprecedented surge in high-pressure research that greatly improved our fundamental understanding of materials under high compression. The research has benefited from the integration of high-pressure, high-temperature instrumentation and analytical probes at synchrotron facilities. Indeed, the integration has gone a long way. During the first-generation approach in 1980's, high-pressure researchers brought the entire experimental setup and high-pressure apparati to an empty synchrotron station. Experiments were restricted to simple ones that could be assembled and dissembled within very limited beam time allotment. During the second generation since 1990's, dedicated high-pressure beamlines established permanent setups that allow users to conduct sophisticated high-pressure experiments efficiently. This mode of operation has been very successful, but also have noticeable shortcomings. Most significantly, many cutting-edge synchrotron techniques that have been advancing rapidly at specialized beamlines are unavailable at dedicated high-pressure beamlines which must consider their primary role in supporting routine, general, high-pressure operations. The development or usage of novel high-pressure techniques at specialized beamlines are left in the hands of high-pressure researchers and beamline scientists who are facing the insurmountable barrier of adapting the high P-T conditions to the beamlines analogous to the first generation mode 20 years ago, except it is even harder now due to the sophistication of the advanced beamlines. Progressing to the next-generation operation, we are organizing the High Pressure Synergetic Consortium (HPSynC) at APS. HPSynC is equivalent to a full beamline team of five scientists/engineers who, working with high-pressure researchers and beamline scientists, are focusing on the integration of novel high-pressure synchrotron techniques at all specialized beamlines without the usual burden of construction and management of a specific beamline. The HPsynC concept is beyond the normal "extreme environment" infrastructure that is limited to sharing gas-filling equipment, sample preparation laboratory, diamond cells, etc. Including these functions, the main role of HPSynC is to facilitate the next level of scientific and technical integration. The HPSynC staff who have the scientific agendas and technical know-how will improve both high-pressure apparati and beamline parameters interactively to optimize the extraordinary capabilities and resource of the synchrotron facility for novel high-pressure experimentation. To retrofit the HPSynC concept to the existing APS CAT infrastructure is feasible but awkward. ERL has an advantage to adopt the concept at the beginning. Extraordinary scientific advances can be achieved with such facility-wide approach and team effort.A Short Overview of the Present Success and Limitations of using a 3rd Generation Synchrotron Source to Measure the Phase Diagram of low-Z Systems at very High Pressure: Would an ERL X-Ray Source Enable Much Progress and How?
Paul Loubeyre Départment de Physique Théorique et Appliquée, CEA. France Over the past ten years, interesting results have been achieved in the determination of the structural and dynamical properties of low-Z systems by optimising the use of the diamond anvil cell in front of large facilities. More particularly, by using the x-ray beam of the 3rd generation synchrotron source. The strategy for progress has relied on improving the three components of such experiments, that is : the sample quality; the characteristic of the beam to reduce the signal to background ratio; the most adapted detector for data collection. That will be illustrated on three examples: the determination of the equation of state of hydrogen by single-crystal x-ray diffraction 1)2); the determination of the structure factor of fluid oxygen and water at high pressure3); the inelastic x-ray scattering data of the sound velocity of water in the THz frequency range4). The determination of the equation of state of hydrogen somehow exemplifies the need to improve the performance of the synchrotron x-ray beam in order to achieve new measurements. The progress has followed a staircase evolution essentially mapping the improvements of the x-ray technique and beam: energy dispersive x-ray scattering (on ID09) , angle dispersive x-ray scattering single crystal (on ID30) , micro-focus angle dispersive single-crystal X-ray scattering (on ID27). The structure of phase I and II have been disclosed. The structure of phase III is within reach. Yet, the ultimate goal is to determine the structure of metallic hydrogen . That should certainly require a significant jump in the x-ray brightness and source size. Such a beam could be obtained by an ERL synchrotron x-ray source. Also, metal hydrogen could probably be observed more easily in a transit time mode by coupling a magnetic pulse, a temperature pulse or a light pulse. But then, the necessity to collect the X-ray data in less than a short time scale will also require the high flux of the ERL synchrotron source.The dynamical structure factor of molecular fluids is essential to understand the evolution of a molecular fluid such as polymerisation, dissociation and ionisation. The problem here is to have enough x-ray flux to be able to collect inelastic x-ray data in a reasonable time. This is all the more important at very high P-T conditions where it is difficult to confine the sample. The use of an ERL synchrotron source will enable to perform the measurements with a reduction of at least one order of magnitude in the accumulation time and also with a better energy resolution. References: 1. X-ray diffraction and equation of state of hydrogen at megabar pressures. P.Loubeyre, R.LeToullec, D.Hausermann, M.Hanfland, R.Hemley, H.K.Mao and L.Finger, Nature 383, 702 (1996) 2. Neutron and x-ray diffraction study of the broken symmetry phase transition in solid deuterium. I. Goncharenko and P.Loubeyre. Nature 435, 1206 - 1209 (2005) 3. Quantitative structure factor and density measurements of high-pressure fluid in diamond anvil cells by x-ray difffraction: argon and water. J.Eggert, G.Weck, P.Loubeyre and M.Mezouar, Phys. Rev. B.65, 174105 (2002) 4. Pressure Evolution of the High-Frequency Sound Velocity in Liquid Water, M.Krisch, P.Loubeyre, G.Ruocco et al , Phys. Rev. Lett. 89, 125502 (2002)New Opportunities for High Pressure and Temperature Experiments using an Energy Recovery Linac
Wendy Mao Los Alamos National Laboratory High pressure can induce dramatic changes in materials, and synchrotron radiation provides a powerful probe for investigation of this behavior. Recent development of the panoramic diamond anvil cell with x-ray transparent gaskets has extended the energy window and led to exciting advancements in high-pressure synchrotron x-ray spectroscopic techniques between 5-15 keV at high pressure and ambient temperature. However, these experiments are photon intensive and require significant x-ray exposure times which severely limit the ability to make measurements at simultaneous high temperature. It is extremely challenging to maintain temperature and sample configuration stability for measurements which take more than 30 minutes. A much brighter source like the proposed energy recovery linac would enable the collection of spectra via a suite of synchrotron x-ray spectroscopy methods (e.g. nuclear resonant inelastic x-ray scattering, x-ray raman spectroscopy, x-ray emission spectroscopy) at high pressure and high temperature in reasonable counting times. It would also facilitate measurements to much higher pressures on smaller samples and high resolution inelastic scattering experiments (e.g. phonon inelastic scattering measurements on Fe to core conditions). This opens the exciting possibility to measure key materials properties in situ at simultaneous high pressure-temperature for understanding behavior in the deep Earth. Materials Chemistry at High Pressure and High Temperature
Workshop on High Pressure Research Energy Recovery LINAC Project at CHESS
Paul F. McMillan
Department of Chemistry and Materials Chemistry Centre, University College London, 20 Gordon Street, London WC1H 0AJ, UK
also at: Davy-Faraday Research Laboratory, The Royal Institution of Great Britain, 21 Albemarle Street, London W1X 4BS, UK
We use a combination of laser-heated diamond anvil cell (DAC) and "large volume" press (multi-anvil, piston cylinder) techniques to synthesise and study new materials formed from chemical precursors at high pressure and high temperature. Studies are being carried out in various systems: transition metal nitrides (high hardness; superconductors); light element solids (B6O -B6N: wide-gap semiconductors; superhard); layered and dense CxNy phases; (Si,Ge,Ga) nitride and oxynitride spinels. We are also exploring the phenomenon of density-driven liquid-liquid phase transitions and polyamorphism, especially among amorphous semiconductors.
Transition metal nitrides and carbides (TiN, WC etc.) are well-known high-hardness materials. Much is known about their mechanical engineering parameters, but their microscopic properties have generally not been determined. We have studied the bulk modulus for several metallic nitrides using synchrotron X-ray techniques in the diamond anvil cell. Because the materials are highly incompressible, we must obtain data to very high pressure: sample sizes are small, and intense focused synchrotron X-ray beams are necessary. Compressibility determinations for light element phases (B6O -B6N) are even more demanding: these materials are "superhard" and the X-ray scattering is very weak. Determining the phonon spectra and Gruneisen parameters of transition metal nitrides is important for understanding and predicting their superconductivity: several of these phases (cubic NbN; hexagonal d-MoN) are relatively high-Tc materials (15-19 K). We currently do this in the laboratory via Raman scattering in the DAC: new experiments using inelastic X-ray scattering will provide a more complete picture of the phonon dispersion relations. Finally, the high-P,T phase relations of transition metal nitrides and B-N-C-O systems are almost completely unknown. We have been using laser heating experiments in the DAC, combined with multi-anvil experiments at lower pressures, to explore the phase relations under high nitrogen pressures. Intense synchrotron X-ray beams are necessary to probe the small samples within the laser-heated spot, or to penetrate the capsule assemblies in the large volume experiments.
Spinel-structured nitrides in the Si3N4 -Ge3N4 system were first reported in 1999, and there has been considerable effort devoted to synthesising and characterising these new solid state materials. Recently, the attention has switched to (Si,Al)- and Ga-containing oxynitride spinels. The new materials have high hardness and are wide direct-gap semiconductors. Now we are beginning to explore and understand the defect chemistry of these new phases, that involves vacancy formation on both cation and anion sites: this determines the optoelectronic properties. In situ high-P,T experiments are necessary to design the best synthesis routes and recovery strategies: synchrotron-based X-ray spectroscopy and diffraction are needed to study the optical properties and details of the crystal structure. In situ experiments in large volume devices are now being designed to study the formation kinetics of the new nitride and oxynitride spinel phases.
Ti3N4 is a transition metal nitride that has been predicted to be stabilised in the spinel structure at high pressure. We have investigated formation of Ti3N4 from "amorphous" Ti(C,N,H) chemical precursors obtained from reactions of organometallic species with ammonia, under high-P,T conditions. The resulting materials appear to be nanocomposites with nanocrystalline TiN embedded in the amorphous matrix. EXAFS experiments indicate that the matrix has a highly-defective structure based on the rocksalt TiN form, but with 40-50% vacancies on cation and anion sites. New nanocomposite materials are expected to result from various high-P,T treatments from the precursor compounds.
We are exploring the phenomenon of "polyamorphism" or liquid-liquid phase transitions occurring as a response to pressure and temperature in various systems, especially amorphous semiconductors. Amorphous Si and Ge have a negative initial melting slope indicating a likely melting curve maximum in the negative pressure regime (under tensile strain). Applying a two-state model to the liquid to understand the anomalous densification results in appearance of a critical point followed by a line of density-driven liquid-liquid phase transitions in the supercooled liquid regime. The transitions occur between low- and high-density liquid phases (LDL, HDL), that become amorphous solids (LDA, HDA) below the glass transition. We studied the compressional behaviour of a-Si by laboratory Raman spectroscopy and electrical conductivity, combined with synchrotron amorphous X-ray diffraction and MD simulations. The LDA-HDA transition is reversible and has the character of a first-order thermodynamic phase transition between semiconducting and metallic amorphous forms of the element: we can extract the Si coordination changes in the two amorphous polyamorphs from the X-ray data combined with the MD simulation results.
References:
McMillan PF; "New Materials from High Pressure Experiments", Nature Materials, 1, 19-25 (2002)
Soignard E, McMillan PF, Chaplin TD, Farag S, Bull CL, Somayazulu M, and Leinenweber K; "High-pressure Synthesis and Study of Low-compressibility Molybdenum nitride (MoN and MoN1 -x) Phases", Phys Rev B, 68, 132101-4 (2003)
Machon D, Daisenberger D, Soignard E, Shen G, Kawashima T, and McMillan PF; "High Pressure-high Temperature Studies and Reactivity of g-Mo2N and d-MoN", phys. stat sol, in press (2006)
Shebanova O, McMillan PF, and Soignard E; "Compressibility Measurements and in situ Raman Scattering Studies of Vibrational Excitations in High-hardness Cubic Nitrides TiNx and g-Mo2N at High Pressure", High Press Res, in press (2006)
Soignard E, Machon D, McMillan PF, Dong J, Xu B, and Leinenweber K; "Spinel-structured Gallium Oxynitride (Ga3O3N): An Experimental and Theoretical Study", Chem Mater, 17, 5465-5472 (2005)
Soignard E, and McMillan PF; "Defect Chemistry in g-Si3N4 and g-Ge3N4 Spinel Nitride Phases Probed by Raman Scattering in the Laser-heated Diamond Anvil Cell", Chem Mater 16, 3533-3542 (2004)
Machon D, McMillan PF, Dong JJ, and Xu, B; "High-pressure Study of the b-to-a Transition in Ga2O3", Phys Rev B, 73, 094125 (2006)
Wilson M, and McMillan PF; "Crystal-liquid Phase Relations in Silicon at Negative Pressure", Phys. Rev. Lett., 90, 135703-7 (2003)
McMillan PF, Wilson M, Daisenberger D, and Machon D; "A Density-driven Phase Transition Between Semiconducting and Metallic Amorphous Polymorphs of Silicon", Nature Materials, 4, 680-684 (2005)
Hector AL, Jackson AW, McMillan PF, and Shebanova O; "Amorphous and Nanocrystalline Titanium Nitride and Carbonitride Materials Obtained by Solution Phase Ammonolysis of Ti(NMe2)4", J Solid State Chem, 179, 1383-1393 (2006)
McMillan PF; "Polyamorphic Transformations in Liquids and Glasses", J Mat Chem, 14, 1506-1512 (2004)
Structures of Solids, Fluids, and Glasses at High Pressures and Modest Temperatures Produced by Dynamic Compression
W. J. Nellis Harvard University The capability to perform x-ray scattering experiments with sufficiently high-intensity and short pulse duration means that structures of materials compressed dynamically could be determined in situ at extreme conditions. Extremely little is known about such structures because it has not been generally possible to perform such experiments. Thus, there are great opportunities in this virtually untouched area, particularly because pressure, density and temperature can be tuned by a combination of shock and isentropic compression. Because x-ray beam size is relatively small, a relatively small two-stage gun (~3 m long) is sufficient for coupling with the accelerator. Because of the short time scales, phases are generally defected fine-grained solids (including nanocrystalline materials), glasses, and fluids. The prime goals are radial distribution functions of glasses and fluids, crystal and defect structures of solids, and phase transitions and their dynamics, all in low-Z as well as higher-Z materials over a substantial range of pressure, density and temperature. The greatest opportunities are in condensed matter at conditions from near ambient up to pressures of a few 100 GPa (1 Mbar), densities up to ten times liquid in the case of hydrogen, and temperatures up to a few 1000 K. The physics of hydrogen at very high pressures is the last frontier of the elements. Structures of dense hydrogen at modest temperatures should be measured in the regime in which zero-point energy of the proton is comparable to the binding energy of the molecule and thermal energy is small compared to both, which effects whether the system is solid or liquid and monatomic or diatomic. The prototypical issue is measuring melting temperature versus pressure, which is predicted to have a maximum near 100 GPa and 1000 K related to quantum effects. Melting would be determined by the transition from a defected lattice to the radial distribution of a fluid. Such structural determinations are expected to provide information on how molecular dissociation effects melting. If the line of metallization intersects the melting curve, a key issue is the nature of the many-body system in which melting, metallization, and possibly dissociation occur simultaneously in a system in which both electrons and protons might be quantum in nature? Another question is how metallization continues from the fluid into the solid. More than 150 extrasolar giant planets have now been observed with masses mostly in the range 0.5 to 5 MJ, where MJ is the mass of Jupiter. These Jovian planets are composed primarily of dense fluid hydrogen. The only experimental technique used thus far to measure properties of hydrogen at both pressures and temperatures in deep planetary interiors is dynamic compression. To understand the natures of these 150 planetary interiors, the dependence of the dissociative phase transition on pressure and temperature should be determined by looking for fluid-fluid phase transitions in measured radial distribution functions. For "hot" and "cold" Jupiters, pressures of interest are 50 to 500 GPa and temperatures of 1,000 to 20,000 K. The Hugoniot of liquid D2 goes right through this regime. The melting curve of hydrogen near 100 GPa, discussed above, is important for understanding interiors of cold Jupiters. Pressures and temperatures in deuterium-tritium fuel pellets in inertial confinement fusion (ICF) traverse this same regime of pressure and temperature in giant planets. Thus, what is learned for purely scientific reasons about giant planets would provide assistance to an R&D fusion-energy project of ever growing national importance. Several other long-standing issues in planetary science would be resolved. For example, radial distribution functions of water, ammonia, and synthetic Uranus could be measured at densities and temperatures in the interiors of Uranus and Neptune and compared with theoretical predictions. The high-pressure phase diagram of Fe has been unresolved for years and could determined by structural determinations of solids and of the molten fluid at pressures and temperatures up to those in the Earth's deep core (300 GPa and 6000 K). Interesting new materials have been synthesized at extreme dynamic conditions and their structures are unknown. For example, Gd3Ga5O12 (GGG) is less compressible than diamond above 170 GPa shock pressure. GGG was found using a simple theoretical predictor of where to look systematically for such materials. In this case, for example, the structure of the high-pressure phase above 170 GPa and the radial distribution function of the amorphous phase between 70 and 120 GPa should be determined. For shock pressures up to 70 GPa, it should be determined on a microscopic scale how this high-strength oxide transitions from uniaxial compression at lower pressures to isotropic compression at higher pressures. It is quite possible that other materials might be found that are less compressible and harder than diamond. One "Holy Grail" of high-pressure research is to retain materials metastably at ambient that are synthesized at high pressure. X-ray scattering might be used to learn the nature of interatomic bonding required to bind materials into a high-pressure phase so that they are recovered from high pressures. Metastable solid metallic hydrogen is the paradigm in this regard because of its numerous scientific and technological applications, depending on the degree to which it is metastable.Short Intermediate Range Structure at High Pressure and Temperatures
John Parise SUNY at Stony Brook The analysis of Bragg scattering and the fitting of models to this scattering is the primary means of validating and determining structure models for condensed matter. The scattering process is well known, and over a century's success in determining small molecule and protein structures, and several Noble prizes, testimony to the power of crystallographic techniques. While comparison of observed and calculated single crystal intensities is the most reliable means of model validation, modern computation methods have greatly expanded the role of powder methods in the analysis materials with long range (> 2 nm) structure. The atomic structure of nano-phase materials, by definition, can not be analyzed by reference to the sharp Bragg diffraction features alone - there are none. Instead, the short (1 - 5 Å) and intermediate (1 - 2 nm) range order give rise to broadened diffraction features and to an increase in the elastic diffuse scattering under and between Bragg peaks. For 1-D powder diffraction data the Fourier transform of the total elastic scattering (Bragg + diffuse) is the pair distribution function (PDF); the scattering power and number density weighted distribution of interatomic distances in the sample. In order to be able to integrate over a particular range of distances, to determine coordination numbers for example, the Fourier transform must be carried out on the total elastic scattering, normalized, and taken to sufficiently high Q (=4πsinθ/λ) to minimize Fourier termination ripple. Further, care must be taken to eliminate inelastic scattering. While these requirements are straightforward for samples at ambient conditions, they represent challenges for high pressure data, chiefly because of contributions from the sample environment, pressure cells for example. For large volume high pressure devices the use of tight diffracted beam collimation and point counters, or radial collimation and linear position sensitive detectors are viable options. Uncollimated beams and area detectors with diamond anvil cells will remain the workhorse beamline setup however, and we have been working to make high pressure PDF measurements reliable. Several trial experiments on crystalline, nano, liquid and glassy materials at high pressure demonstrate the power of high energy X-ray scattering, at times combined neutron scattering with isotope substitution as a means to garner valuable extra information, in solving the short and intermediate range structure of "crystallographically challenged" materials at high pressures. Examples include the structural transformations of nano-FeS and glassy GeSe2 to 10 GPa. In some cases these experiments, carried out at 100 keV with a beam focused to 20 μm with Si saw tooth refractive lenses, are at the limit of what it is possible with an APS undulator.High-Pressure Diffraction and 4th Generation Sources
Malcolm I. McMahon School of Physics and Centre for Science at Extreme Conditions, The University of Edinburgh, Edinburgh EH9 3JZ, UK The last 15 years have seen an unprecedented growth in the number of detailed structural studies conducted at high pressure. In that time, new experimental methods, and the advent of 3rd generation light sources such as the ESRF, APS and Spring-8, have seen detailed structural refinements extended to above 200GPa, and the discovery of ever more complex structure types. In many cases, these structural studies have been accompanied by other x-ray based techniques, such as inelastic x-ray scattering, x-ray raman scattering and x-ray nuclear scattering, which have all been made possible by the use of 3rd generation sources. The construction of 4th generation sources, such as the Cornell ERL, will offer ultra-high brilliance beams of hard x-rays that are only a few microns in diameter, and which will also have very low emittance, transverse coherence, and very short pulse lengths. In this talk I will illustrate current state-of-the-art x-ray diffraction and scattering studies at 2nd and 3rd generation sources, using some recent results on incommensurate Rb, Sc and S at high-pressure. I will then look to the kinds of new science that might be possible on such systems using the ERL.High Pressure and Conventional Macromolecular Crystallography using Ultra-short Wavelengths. The Case of the Cornell ERL Source
DR. Fourme1, E. Girard1, I. Ascone1, R. Kahn2, A-C Dhaussy3, and M. Mezouar4 1Synchrotron SOLEIL, BP48, Saint Aubin, France2IBS, Grenoble, France
3ENSICAEN, Caen, France
4ESRF, France The combination of macromolecular crystallography (MX) and pressure perturbation was pioneered nearly 20 years ago using Be cell. This device was used to determine 3D structures of small proteins ([1],[2]). Diamond anvil cell (DAC) extended the pressure domain beyond 200 MPa. It was used first, in combination with a 2nd generation SR source, for compressibility measurements on lysozyme crystals without subsequent data collection [3]. Recent progress [4] include the use of ultra-short wavelength X-rays emitted by in vacuum undulators at a high energy 3rd generation SR source (ESRF). Data collection are performed at room temperature using special sample mounting techniques. The crystal is translated during data collection in order to irradiate successively fresh zones. The quality of diffraction data recorded on the ESRF ID30/ID27 beamlines can meet usual standards [5]. The 3D structures of proteins (monomeric, dimeric and tetrameric) and of a virus have been refined both at atmospheric and at high pressure. Accordingly, high pressure macromolecular crystallography (HPMX) can now be considered as mature. Beyond classical studies such as the molecular basis of the adaptation of life to extreme conditions, exploring the energy landscape - and accordingly most of the biologically relevant conformational space of the protein (or other macromolecules) - is probably the major interest of combining pressure perturbation with structural methods. Pressure paves the way to trap and study, in solution [6] or in the crystalline state [2, 7], substates and high energy conformers of biological significance. Indeed, prospects are bright [7]. HPMX requires an intense, parallel and tightly collimated (5-50 microns) hard X-ray beam. (wavelength 0.03-0.04 nm). The extremely high brilliance of the ERL source in this wavelength range will be ideally suited for HPMX using either monochromatic or limited-bandpass Laue techniques. For the scientific case of the proposed Cornell ERL source, we underline that what was learnt from HPMX studies could benefit to the whole field of MX. On the one hand, the unusual experimental conditions used for HPMX data collection ensure high data collection efficiency (DCE). This is also valid for conventional (i.e. at atmospheric pressure) MX, with the prospect to acquire routinely data of unprecedented quality [5]. Second, pressure is a way to improve order in pre-existing crystals [8], and this is of general interest for MX. References: 1. C. E. Kundrot and F. M. Richards; J. Mol. Biol. 193, 157-170 (1987) 2. P. Urayama, G.N. Phillips and S.M. Gruner; Structure 10, 51-60 (2002) 3. A. Katrusiak and Z. Dauter; Acta Cryst. D 52, 607-608 (1996) 4. R. Fourme, R. Kahn, M. Mezouar, E. Girard, C. Hoerentrup, T. Prangé, and I. Ascone; J. Synchrotron Rad. 8, 1149-1156 (2001) 5. R. Fourme, E. Girard, R. Kahn, I. Ascone, M. Mezouar, A. C. Dhaussy, T. Lin, and J. E. Johnson; Acta Cryst. D 59, 1914-1922 (2003) 6. K. Akasaka; Biochemistry 42 10875-10885 (2003) 7. R. Fourme, E.Girard, R. Kahn, A-C Dhaussy, M. Mezouar, N. Colloc'h, and I. Ascone; BBA 1764, 384-390 (2006) 8. E. Girard, R. Kahn, M. Mezouar, A-C Dhaussy, T. Lin, J. E. Johnson, and R. Fourme; Biophysical J. 88 3562-3571 (2005)
Imaging Opportunities for High-pressure Research
D. Walker Lamont-Doherty Earth Observatory, Columbia University, Palisades NY 10964 Interesting physical chemistry insight often arises from observations of reaction zones between chemically incompatible substances. Geologic skarns in nature and corrosion in the lab are examples of such spatially-resolved reaction zones. Observation of the growth of new intermediate phases and of the compositional shifts of adjacent phases, are extremely useful for defining phase equilibria and saturation boundaries in an efficient manner. Recognition of the spatial dimension to the reaction information provides considerably more insight than characterization of bulk-mixture reactions alone. In natural rocks from depth, all scales from map pattern, to mesoscopic, to hand-specimen, to microscopic, and to grain boundaries provide features of interest after the reaction has been exhumed to the Earth's surface. However in the laboratory under extreme conditions such as high pressure, spatial scales are much more limited towards the lowest end of the natural range. The limits are the small physical dimensions of the reaction chamber and the limited times available for mass transfer to generate reaction encrustations physically large enough to be observable. Mother nature is more patient but has a rather limited palette of compositions that can encounter one another in interesting reaction situations - which are generally not observable in real time.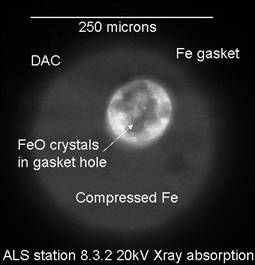
Understand Condensed Matter at Extreme Conditions: by Integrating Dynamic and Static Compression Methods
Yogendra M. Gupta Department of Physics and Institute for Shock Physics, Washington State University The combination of shock wave and static pressure experiments has been used for more than 50 years to gain insight into the response of condensed matter at extreme conditions. While both these approaches result in large compression of matter, there are significant differences between the states of matter achieved using these two methods: uniaxial strain versus nearly hydrostatic compression; large temperature rise versus isothermal compression; and very short time response versus very long time response. Depending on the magnitude of peak stresses achieved in shock compression experiments, one or more of these differences may play a significant role in comparing the two sets of results. At high stresses (well above a Mbar), the temperature rise under shock compression leads to thermodynamic states that are quite different from the isothermal compression under static pressure loading. At lower stresses (below a Mbar), deformation and kinetic issues can result in significant differences in matter compressed statically and using shock waves. This talk will give an overview of shock wave compression issues along with representative examples of some recent achievements. Some new developments will be indicated with a particular emphasis on ramp wave loading resulting in quasi-isentropic compression. Ramp wave loading experiments have two important benefits: to provide thermodynamic states that are closer to isothermal compression, and to span large regions of thermodynamic space that are not accessible by either shock wave or static pressure experiments. Thus, dynamic compression experiments, due to recent advances in pulsed power technology and lasers, are now very versatile. However, to achieve good accuracy in the results and to gain detailed insight into the material response will require careful and systematic wave propagation analysis; a small price to pay for very significant payoff. With increased versatility of dynamic compression experiments, there is an ever-increasing impetus to combine dynamic and static compression methods to better understand matter at extreme conditions. Static compression experiments have some inherent benefits: separation of compression, deformation and temperature. Perhaps, the most significant benefit of static pressure experiments is that they are more amenable to "microscopic" examination using a diverse array of methods available at national and international facilities like CHESS, APS, ALS, and LCLS. Advances in dynamic compression drivers, DAC capabilities, and national facilities for improved probing of matter point to an exciting future for understanding condensed matter at extreme conditions. In my opinion, two challenges are paramount: how to achieve constructive synergy between national and international facilities; and how to ensure that the whole is greater than the sum of the parts. Finally, deep scientific understanding will require that theory and experiment be integrated in an effective and meaningful manner.Poster Abstracts
How to Make Full use of 100 fsec Pulses in a Diffraction/XAFS/Heating Experiment
Keith Brister Northwestern University 100 fsec x-ray pulses present a opportunity to sense the instantanious state of a high pressure experiment: a 100 fsec pulse extends 30 microns along the beam path, matching the size of a typical diamond anvil cell sample chamber. This spatial resolution combined with the high repetition rate allows extension of high pressure techniques developed by Brister and Bassett, Rev Sci Instrum 66, 2698 (1995) for carbon dioxide laser pulses and energy dispersive diffraction by using modern detectors, lasers, and electronics. Laser heating pulses allow heating the sample to a higher temperature than can be achieved using CW techniques while area detector data can be sorted based on measured experimental conditions. The energy recover linac combined with avalanche photo diodes and pixel array detectors point to an exciting future for diamond anvil cell experiments.Exploring High Energy Density Matter at Pressures Ranging from a few Kilabar to Several Gigabar
Gilbert W. Collins1, Raymond Smith1, Damien Hicks1, Jave Kane1, Jon Eggert1, Peter Celliers1, Marina Bastea1, Yogi Gupta2, James R. Asay2, Paul Loubeyre4, Stephanie Brygoo4, Tom Boehly5, David Meyerhoffer5, Raymond Jeanloz6, Ryan McWilliams 6, David Bradley1, and Dan Kalantar1
1Lawrence Livermore National Laboratory
2Washington State University
3Los Alamos National Laboratory
4CEA, France
5Laboratory for Laser Energetics
6University of California, Berkeley
A new generation of materials experiments at high pressures and densities is now possible due to a variety of high energy density (HED) facilities and new compression techniques. Shock and shockless compression experiments can now produce material states from kilobar to gigabar pressures with timescales ranging from picoseconds to microseconds. While the production of truly novel material states is well underway, the careful inspection of these states is still maturing. The marriage of these new HED capabilities with advanced light sources will enable the detailed characterization of these new states. Described first are several recent HED experiments to highlight the extreme material states produced with HED facilities. A few ways that advanced light sources can revolutionize the characterization of such HED matter are suggested.
Laser shock compression experiments have measured the insulator-conductor transition and the high-pressure equation of state of several low Z materials (C, H2O, SiO 2, H2, D2, He) from Kbar to 10's of Mbar. These experiments enable us to recreate the core states of giant planets and low mass stars in the laboratory. For example, recent experiments show shock compressed He becomes an electronic conductor at pressures significantly lower than expected albeit at higher shock pressures and temperatures than hydrogen. Another interesting example is diamond, whose compressibility has recently been measured to over 30 Mbar. The diamond melt curve was recently discovered to have a negative dP/dT along the Hugonoit. Moreover, shocked diamond transitions from an insulator to an electronic conductor upon crossing the melt. In addition to shock experiments, shockless compression experiments are being used to explore "low temperature and high pressure" multi-phase diagrams and phase transition kinetics with loading rates ranging from 106 to 108 s-1. This technique has been used to determine the quasi-isentrope of Al to near 1 Mbar and solid-solid phase boundaries and transition kinetics for Fe, Bi, and Ce.
X-ray diagnostics are often used to explore these HED states, ie the compressibility, lattice structure, temperature, and spatial structure. Often what limits the accuracy of these x-ray measurements is the brightness and spectral quality of the source. We show a couple of examples where an improved x-ray source could dramatically change the accuracy of such measurements.
Investigations of Earth Electrochemistry
Abby Kavner University of California, Los Angeles Electrochemical reactions, which involve the transfer of charged species during a chemical reaction, play an important role in governing the evolution of the Earth and planets. For example, the evolution of the core/mantle system and ongoing reactions between the core and the overlying reactions are likely electrochemical in origin. In addition, deep Earth oxygen fugacity - which helps determine deep mantle mass transport, heat transport, and electrical conductivity - can be understood in terms of the electrochemical potential of the deep Earth. Element cycling (e.g. Fe, C, S, O) throughout the Earth's atmosphere, hydrosphere, biosphere and tectosphere also involve charge-transfer reactions, and their behavior is therefore governed by electrochemical principles. In the laboratory, application of an electric field can be used to drive electrochemical reactions, and to manipulate oxygen fugacity in situ. The resultant chemical and physical behavior can be examined by a variety of techniques, including microscopy, mass spectrometry, X-ray diffraction and micro X-ray absorption edge spectroscopy. If electrochemical reactions generate unique signatures, then some geochemical and geophysical observations of the Earth may be shown to correspond to specific electrochemical behavior. In this poster, we present recent results from ongoing studies at UCLA examining geochemical signatures of electrochemical processes in Earth materials. In a study on the aqueous electrodeposition of Fe metal from an Fe(II) chloride solution, a voltage-dependent stable isotope fractionation was discovered (Kavner et al., Geochim. Cosmchim. Acta, 2005). We present new results showing similar-type isotope fractionations during Zn electrodeposition. We examine the results in terms of two competing hypothesis for the isotope-selective deposition phenomenon: electron-transfer limited behavior, and mass-transfer limited behavior at an electrode. Electrochemical reactions can also be driven at high pressures. The effect of pressure on reaction rate provides information about reaction volumes in the high pressure phases. Redox reactions in AgI, an ionic salt, can be pushed and pulled at electrodes attached to a power supply, in situ at high pressures. We show the results of recent experiments investigating the rate of Ag deposition and iodide oxidation as a function of pressure, phase, and applied voltage in the hydrothermal diamond anvil cell.Applications of ERL X-Rays in Dynamic Compression of Condensed Matter
Sheng-Nian Luo, Damian C. Swift, and Dennis L. Paisley P-24 Plasma Physics, Physics Division, Los Alamos National Laboratory The ultra-bright and well-controlled ERL X-ray sources supply a powerful tool for time-resolved experiments on condensed matter subjected to shock wave loading at time scales ranging from sub-ps to ms, including diffraction, radiography and spectroscopy in conjunction with conventional shock wave diagnostics. The applications include but not limited to equation of state, phase transitions, plasticity, defects, spall and damage, optical properties, and chemistry. The shock wave loading will mostly utilize direct laser ablation and flyer plates driven by portable lasers in the pressure range of 1 - 500 GPa. Our early efforts will be dedicated to establishing the paradigms for shock experiments using different ERL diagnostics. The pilot experiments on phase transitions using time-resolved ERL X-ray diffraction (TEXD) will be conducted on Gallium to probe shock-induced melting, solidification, solid-solid transition, and their kinetics. Shock-induced decomposition of CaCO3 will be probed using time-resolved spectroscopy at ERL's ultra-fast mode. X-ray radiography will be attempted to investigate spall of single- and bi-crystal NiAl. Transmission spectrum of shocked Al in the ERL X-ray regime will also be measured. When firmly established, we expect to extend these techniques to a wide range of materials and pressures.Al2O3 Incorporation in MgSiO3 Perovskite and Ilmenite
Wendy R. Panero Department of Geological Sciences, Ohio State University First-principles calculations predict that MgSiO3-perovskite dissolves about 15 mol% Al2O3 at the top of the lower mantle, limited by coexistence with Al-rich ilmenite (corundum). The solubility increases with pressure so that the lower mantle is likely undersaturated in alumina in all but the coldest parts of the uppermost lower mantle. The akimotoite-corundum solid solution is highly non-ideal with a symmetric regular solution parameter W=66 kJ/mol per formula unit at the top of the lower mantle. The critical temperature for exsolution of ilmenite is predicted to be 2000 K. The MgSiO3 solution is also significantly non-ideal; assuming only Tschermak substitutions, the value of W=10 kJ/mol per formula unit. Total energy calculations over a range of compositions and several configurations for each composition are based on density functional theory in the local density approximation. The entropy of solution of both structures is nearly ideal on the basis of random sampling of simulations using semi-empirical interatomic potentials.Capturing Transitory States of Reconstructive Phase Transitions in Shock-recovery Experiments
O. Tschauner1,2, S. N. Luo3, P. D. Asimow2, T. J. Ahrens4, and D. C. Swift3 1High Pressure Science and Engineering Center, Department of Physics, University of Nevada Las Vegas2Division of Geological and Planetary Sciences, California Institute of Technology
3Plasma Physics (P-24) and Earth and Environmental Sciences (EES-11), Los Alamos National Laboratory
4Lindhurst Laboratory of Experimental Giophysics, Seismological Laboratory, California Institute of Technology Upon compression many materials undergo major reconstruction of their structure and bonding involving an increase in coordination of constituting atoms and change in bonding-character. While transforming, the materials pass through intermediate states, which are usually to fugitive to be captured and examined. Shock experiments allow in many cases for quenching such intermediates structural states. We review recent results on intermediate states for carbon phases. First we discuss an initial state of C60 polymerisation, then an example of extreme compaction of a C60-like cage structure. C60 forms intermolecular bonds by 2+2 cycloaddition.This process can be induced by irradiation with light of suitable energy at ambient pressure leading to formation of dimers and oligomers. Cycloadditon also occurs at elevated pressures and temperatures where it yields itinerant polymers. Pressure induced polymerisation is selective with respect to rotational orientation of the C60 molecules. At ambient pressure molecular C60 exhibits partial rotational ordering at 300 K. We performed X-ray diffraction studies on C60 retrieved from laser driven hypervelocity shock experiments and find rotational ordering enhanced even at 300 K. Ordering occurs in the (111) plane and is accompanied by slight rhombohedral distortion of the cubic metric. Further we observe diffuse scattering at Q-values between the (220) and (311) reflections which is interpreted as result of random polymerisation of C60 molecules in the (111) plane. In consequence, the remarkably high degree of rotational ordering in shocked molecular C60 is interpreted as result of enhanced lattice strain in the (111) plane induced by random polymerisation. The present findings provide insights into the mechanism of pressure-induced polymerisation of C60 and their relation to rotational ordering. At much higher pressures and temperatures, C60 polymers collapse into disordered networks. Ultrafast dynamic compression and decompression allows for quenching a crystalline dense polymer at the brink of this density-driven breakdown: We describe an anisotropic 3D polymer cage structure equivalent to an extremely compact C60 polymer where random displacements of carbon atoms maximize bond distances. This material has been synthesized by laser-driven hypervelocity shock out of graphite. Thus, it represents a structural crossing point between graphite interlayer bridging and C60 polymerization as two paths of diamond formation out of low-density carbon phases.
ERL and Internal Resistive Heating for the DAC
Chang-Sheng Zha1,*, William Bassett2, Kenji Mibe2,3, Oliver Tschauner4 1Cornell High Energy Synchrotron Source, Cornell University2Department of Earth and Atmospheric Sciences, Cornell University
3Earthquake Research Institute, University of Tokyo
4High Pressure Science and Engineering Center and Department of Physics, University of Nevada, Las Vegas
*Present address: Carnegie Institution of Washington, Geophysical Laboratory An internal heating system has been developed for the diamond anvil cell (DAC). With it samples 10 microns in diameter can be subjected to pressures up to 80 GPa and 1900K. These temperatures and pressures are higher than can be achieved with external heating and are more uniform and constant than can be achieved with laser heating. X-ray diffraction (XRD) and X-ray fluorescence (XRF) are among the most basic analytical tools for studying samples at these conditions. Although third-generation synchrotron sources provide small, very brilliant X-ray beams, it is beam size and brilliance that limit what can be accomplished. Some important benefits and experiments that can result from combining internally heated DAC and an ERL beam. 1. Increasing experimental conditions to higher pressures and temperatures will require smaller heaters and samples. To gain enough signal intensity and avoid interference from the heater, we need a very brilliant and exceedingly small X-ray beam. 2. Determination of melting pressures and temperatures up to conditions of magma formation within the Earth will be possible by X-ray diffraction in the internally heated DAC using an X-ray beam that is small enough and brilliant enough. 3. Determination of compositions and crystal structures of individual grains of solid phases coexisting with the melt phase will be possible by X-ray fluorescence and diffraction in the internally heated DAC using a very small and brilliant X-ray beam. If the beam is sufficiently small, imaging may be possible by rastering. Determination of elastic properties may be possible by using a nano-size ERL beam to probe individual single-crystal grains in a sample subjected to high pressures and temperatures and to determine their anisotropic elastic strain from their Laue patterns. Accurate equation of state determinations, deviatoric stress, strength measurements, and pressure measurements would all benefit from this information.